Direct Cooling of Ground-State Molecules | Ultracold KRb Molecules
Cold Molecules offer the opportunity to investigate, measure, and control quantum processes that are far more complex than those governing the behavior of atoms. In collisions, for example, atoms are like basketballs, running into one another, bouncing apart, and even forming molecules in ways that are (relatively) easy to observe and explain with quantum theory. Dipolar molecules, on the other hand, collide like American footballs. Angles and spin orientation matter, making it much more exciting to try to predict, observe, and control molecular collisions and reactions. Our group is investigating several different cold molecules (including OH and H2CO ground-state molecules, Sr2, and KRb) with the goals of (1) studying quantum physics with dipolar molecules, (2) performing precision measurements of fundamental physical constants, and (3) controlling ultracold chemical reactions at the quantum level.
Making a quantum degenerate gas with football-shaped dipolar molecules is more complicated than making one from atoms. However, it provides more opportunities to explore a rich set of quantum dynamics. If dipolar molecules are stacked parallel to one another, they repel each other. In contrast, if they are lined up end-to-end, they attract each other. Controlling the orientation of dipolar molecules is the game to play in a dipolar quantum gas.
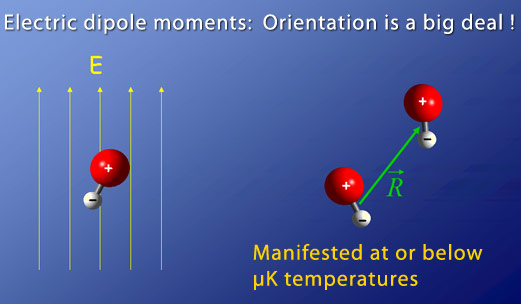
To create a quantum gas of polar molecules, a dense sample of molecules must be cooled to ultracold temperatures — a major technical hurdle. Several approaches exist, but all require solving major problems to advance. However, the payoff for success will be huge. A dipolar molecular quantum gas will be an excellent quantum mechanical model of solid-state materials, allowing the investigation of spin waves, quantum phase transitions, and large interaction strengths. Dipolar molecules should also provide a novel method for studying and controlling quantum information and dynamics.
Cold molecules will also open new possibilities for precision tests of fundamental laws of nature, including
the search for possible time variations of physical constants. While atomic structure is governed basically by
quantum electrodynamics (QED), molecules exhibit more complex structure and dynamics including vibrations and
rotations of their constituent nuclei. These nuclear motions occur inside potentials created by electron motions.
Together they reveal fundamental forces of different origins within one system. For example, one could precisely
measure some vibration frequencies inside a molecule and compare them against electronic transitions that originate
in QED. Such studies may provide some of the best checks one can make on possible variations over time of the
proton/electron mass ratio (mp+/me-) or the fine structure constant ().
Our group has started to explore several different molecular systems and perform laboratory-based precision
measurements to determine time dependence of fundamental constants in the modern epoch. Some of the laboratory-based
measurements can be compared with astrophysically observed molecular spectra, providing a peek into the behavior of these
fundamental constants over the entire life span of the universe. Whatever the group discovers, cold molecules promise
to be a richer system to investigate than atoms, offering powerful new tools to probe the fundamental nature of our world.
Cold molecules are also the key to understanding the quantum mechanics of ultracold chemistry. Our group is exploring several methods for preparing cold molecules in a single quantum state, then transferring them en masse to another quantum state. By controlling molecular quantum states, the group will be able to experimentally observe and control chemical processes (possibly via multiple pathways) in elementary 4-5 body systems that are extremely challenging to model theoretically. The study of molecular collisions and reactions under such well-controlled conditions is a completely new regime for physical chemistry.
The group currently has three major cold molecule projects: (1) precision measurement using (i) directly cooled ground-state molecules (such as OH and H2CO) and (ii) ultracold molecules produced by the pairing of ultracold atoms 1; (2) direct cooling and trapping of ground-state molecules for studies of dipolar collisions at cold temperatures; and (3) explorations of magneto-photo association of ultracold KRb molecules with the aim of achieving a quantum degenerate gas of polar molecules (in collaboration with D. Jin's group).
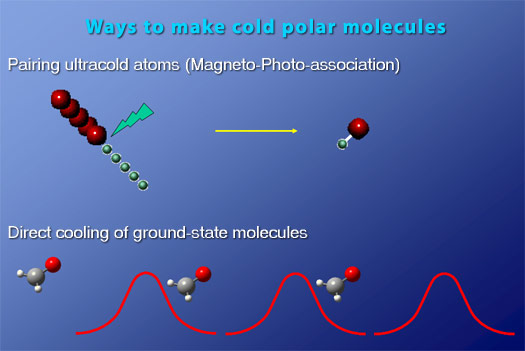
Direct Cooling of Ground-State Molecules
In 2002, our group began cooling studies of highly reactive OH radicals with the goal of making precision measurements
of four specific transition frequencies that have different dependences on the fine structure constant ().
The hope was that precise laboratory measurements could then be compared to similar OH transitions in outer space that took place
billions of years ago in the early universe (but which were only recently detected on Earth). Provided both astronomers and
laboratory scientists could measure at least two of the four transitions precisely enough to detect small differences in
, the value of
today could be compared with its value 10 billion years
ago. If
stayed the same, this result would support the Standard Model; if not, it would support one
of the newer models for the basic structure of matter that predict changes in
over vast spans of
cosmic time.
Our group succeeded in making their precision measurements by using a Stark decelerator (invented by G. Meijer's group and developed in the JILA lab in 2003) to put the brakes on fast-moving OH molecules until they traveled slowly enough through a microwave cavity to be measured. The Stark decelerator could slow these interesting dipolar molecules to "lukewarm" temperatures of about 30 mK. By precisely controlling the molecular beam speed, the group was able to improve the precision measurement of one key transition 25-fold to ±4 Hz, a second key transition by tenfold to ±12 Hz, and two satellite transitions by tenfold to ±10 Hz and ±15 Hz, respectively. Our group is currently awaiting equally precise measurements of 10-billion-year-old OH transitions to know whether the fine structure constant is constant or inconstant.
Our group is currently working with theorists from the John Bohn and Chris Greene groups at JILA on developing more effective cooling methods for ground state molecules. This theory-experiment collaboration has set ambitious goals: (1) figuring out how to cool large numbers of cold OH molecules to ultracold temperatures of less than 0.1 mK, (2) investigating and eventually controlling collisions of OH with other molecules, and (3) in the future, exploring ways to use the strong dipolar interaction in OH molecules as molecular qubits in quantum information processing.
The theorists' first undertaking was a two-year analysis to determine whether OH could be sympathetically cooled with ultracold rubidium (Rb) atoms in a magneto-optical trap (MOT). For sympathetic cooling to occur, the atoms would have to frequently collide, with OH ending up in the same internal state after the collision as before, and colder. In contrast, internal state changes would result in hotter, faster molecules, which would escape from the trap. Unfortunately, the two outcomes occurred with about equal frequency, resulting in "simply pathetic" cooling. It was "back to the drawing boards" for the theorists, who began looking for alternatives to sympathetic cooling for lowering the temperature of large numbers of OH molecules to µK levels.
Credit: Animation by Greg Kuebler
Meanwhile, our group realized that the theorists had stumbled onto a very interesting collision process, in which the dipole moment of an OH molecule would flip its direction in roughly one of every two collisions. And, every time a dipole flip occurred, the molecule flew out of the trap, making it easy to precisely measure the fraction of molecules changing state. This behavior meant the group could study cold OH-OH collisions dominated by strong dipole-dipole interactions as well as other phenomena subject to similar long-range quantum influences.
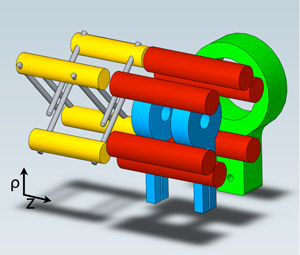
Magneto-electrostatic trap used to confine OH molecules
at 30 mK. The high-current coils (blue) apply a magnetic
quadrupole trapping field while the surrounding
electrodes (red) allow for the application of electric
fields to the trapped OH sample.
So, the group collaborated with theorists to develop a magneto-electrostatic trap for studying cold collisions. In the process, We optimized the Stark decelerator to produce molecules moving slowly enough to trap. Then we coupled the end of the decelerator into a magnetic trap surrounded by electrodes capable of creating both uniform and nonuniform electric fields.
In studies of the new trap, we discovered that an applied electric field not only affected the number of molecules trapped, but also modified the trap itself, making it deeper so fewer molecules could escape. The electric field also changed the oscillation frequency of the OH molecules inside the trap. The magnetic and electric fields exerted comparable forces on the trapped molecules. To understand these unexpected effects, our turned once again to their theory collaborators to help explain how the trap worked on the quantum mechanical level. A better theory allowed our to understand the OH dynamics they observed in their trap, including collisions between OH molecules and nitrogen (N2) molecules that occurred when the N2 molecules were introduced into the trap. Our group was able to extend the lifetime of the new trap and increase the number of molecules confined inside. We are now evaluating ways to study collisions of OH with other polar molecules to see whether such collisions depend on an applied external electric field — a key step in using electric fields to control cold molecule collisions and possibly even chemical reactions.
In the meantime, our group has also begun exploring innovative experimental techniques for cooling cold OH molecules to ultracold temperatures (<1 mK). We are particularly interested in cavity-assisted laser cooling, which provides dissipative cooling that is largely independent of the details of a given molecule's structure when coupling between the molecules and the cavity is strong. Thus far, our group has identified conditions necessary to achieve efficient cooling and experimentally feasible approaches to cooling without loss such as superradiant scattering associated with intracavity molecular self-localization.
Ultracold KRb Molecules
The complicated structures of ordinary "warm" molecules make it difficult to cool them to very cold temperatures. One important method under investigation for creating cold molecules is the use of magnetic Feshbach resonances to make them from cold atoms. Our group is currently collaborating with the Deborah Jin group to use this method to produce cold ground-state KRb molecules with dipolar interactions, starting from a quantum degenerate gas of 40K and 87Rb atoms. In 2007, the Jin group produced more than 30,000 heteronuclear Feshbach molecules at about 120 nK and observed molecules lifetimes as long as 100 ms near the Feshbach resonance.
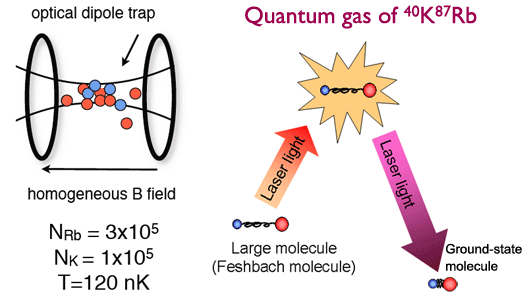
The formation of Feshbach KRb molecules is just the first step in producing stable ground-state molecules, however. Feshbach molecules are large, loosely bound, and form in the highest vibrational state possible of the ground electronic state. Producing deeply bound and stable cold polar molecules requires driving these Feshbach molecules down to the ground state's lowest vibrational level. Fortunately, the relatively long observed lifetimes of the KRb Feshbach molecules bode well for forcing the molecules through this critical transfer.
A recent analysis by the our group showed that femtosecond frequency combs can control this transfer with high precision. The transition process begins with the nuclei of the Feshbach molecules oscillating in a potential well, but spending most of their time near the external vibrational turning points at the walls of the well. An ultrashort laser pulse can pump these molecules into a new excited electronic potential well, where the nuclei begin to vibrate in response to their new environment. If the inner turning points of the new potential well sits directly above the lowest point in the ground potential well, then another laser pulse can dump the molecules directly into the bottom of the ground well, i.e., into their ground states. On the quantum level, there are many small pump/dump steps. With each step, a small fraction of the molecules' wave functions is transferred into the ground state. The molecules are gradually transferred by the cumulative effect of many laser pulse pairs, in a process known as coherent accumulation. For this process to work, however, both the pump and dump pulses must be perfectly synchronized to the quantum behavior of the molecules. Having both the pump and dump lasers be femtosecond frequency combs with exactly matching frequencies accomplishes this synchronization. Even with frequency combs, though, coherent accumulation requires time during which the molecules must not be disturbed by collisions. The 100 ms lifetimes of the cold KRb molecules made by the Jin group should be long enough for the process to work. Our group is currently planning a series of experiments to test their predictions regarding coherent accumulation via a series of pump/dump steps. If successful, this method will result in significant numbers of cold ground-state polar molecules.
Footnote
1For the investigations of the time dependence of nuclear vibrations in ultracold Sr2 molecules, in anticipation of upcoming precision measurements of mp+/me-, see Photo-Association of Ultracold Sr Molecules in the Ultracold Strontium section.