Direct Frequency Comb Spectroscopy | XUV Comb Generation | Laser Stabilization & Comb Characterization| Lab Temperature Data Login |
Since the late 1990s, there has been a remarkable convergence of the fields of ultrafast optics, optical frequency metrology, and precision laser spectroscopy — a convergence that a collaboration at JILA, including the Ye labs, was privileged to help facilitate. A remarkable transformation took place in these fields as unprecedented advances occurred in the control of optical phases ranging from the ultrashort (femtoseconds) to laboratory time scales (seconds). Today, a single-frequency continuous optical field can achieve a phase coherence time exceeding 1 s. This phase coherence can be precisely transferred to the electric waveform of an ultrafast pulse train!
The transformation in ultrafast science began in 1999 when researchers gained control of the frequency spectrum of ultrashort-pulse-mode-locked lasers. With precision laser stabilization, the spectra produced by these lasers manifested as optical frequency combs of discreet, regularly spaced sharp lines, with each line a different color (frequency). By the end of this banner year, the JILA collaboration (including our group) had generated an octave-spanning optical frequency comb. This accomplishment rapidly led to the precision measurement of several optical frequency standards, including an iodine-stabilized HeNe laser operating at 633 nm. A few months later, the collaboration created a direct link between the microwave and optical regions of the electromagnetic spectrum via a broad frequency comb spanning more than 300 THz (an optical octave).
The phase-stabilized-octave-spanning optical frequency comb established millions of precise marks on an optical frequency "ruler." These marks were stable and accurate at the Hz level. Researchers rapidly realized they could be used like a ruler to measure the light emitted by lasers, atoms, molecules, stars, or other objects with extraordinarily high precision. Not surprisingly, optical frequency combs have now become precision measurement tools for frequencies of light from infrared through the far, or vacuum, ultraviolet (VUV) wavelengths. These coherent light-based precision measurement capabilities may eventually be extended to the extreme ultraviolet (XUV) region, where new possibilities and challenges await for precise tests of fundamental physical principles.
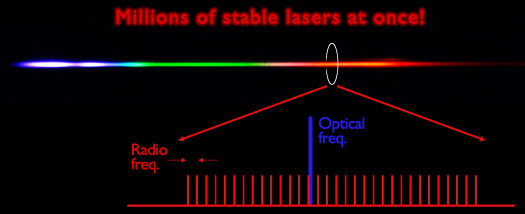
Octave-spanning optical frequency combs enabled revolutionary advances, including accurate phase connections among different parts of the electromagnetic spectrum. For example, it allowed researchers to measure two radio frequencies that described an entire comb and thus precisely determine individual frequencies of millions of optical comb lines. This extraordinary capability has profoundly changed optical frequency metrology (resulting in a transformation in absolute optical frequency measurements), optical atomic clocks, and optical frequency synthesis. The application of optical frequency combs to investigations of ultracold atoms and molecules has resulted in optical spectroscopy, frequency metrology, and quantum control at the highest levels of precision and resolution.
The precision of the comb ruler is directly tied to the type of laser used to make the comb. Researchers use mode-locked lasers that emit pulses lasting millionths of a billionth of a second (femtoseconds) to make combs spanning several hundred thousand to millions of frequencies. The billions of pulses in the pulse train of these lasers all remain phase coherent with each other, providing remarkably sharp profiles of individual frequency comb components, i.e., high spectral resolution. Mode locking refers to how the laser light is formed into pulses. As with all lasers, light is repeatedly reflected within a mirrored cavity. In a mode-locked laser, however, peaks of different frequencies coincide at regular intervals, which are evenly spaced in time. Multiple peaks add up to form very short, very bright bursts of light.
The timing between pulses determines the spacing between the teeth of the comb, or the pulse repetition rate ƒr. The faster the pulse repetition rate, the wider the spacing between the comb teeth, which makes it easier to identify each individual frequency (tooth). The width of each tooth, or frequency line, is determined by the stability of the laser. The most highly stabilized lasers produce the very fine teeth needed to precisely measure specific frequencies or changes in frequency.
Both the spacing between comb teeth and the starting position of the comb, or carrier-envelope phase offset (ƒ0) can be precisely controlled, as verified soon after the discovery of the comb by the JILA collaboration and the group at the Max Planck Institute for Quantum Optics headed by Ted Hänsch. This discovery opened the door to revolutionary advances in basic and applied research on the time-domain characteristics of optical frequency combs. For example, precise control of the pulse repetition rate and the carrier-envelope phase offset has now reached the subfemtosecond regime. This phase coherence can now be routinely maintained for extended periods of time, making it possible to synthesize electric fields at optical frequencies with known amplitudes and phases. These developments recently led to demonstrations of ultrabroad, phase-coherent spectral generation, united time-frequency spectroscopy (including atomic spectroscopy and molecular spectroscopy), coherent control in nonlinear spectroscopy, coherent pulse addition without any optical gain, coherent generation of frequency combs in the VUV spectral regions, and broad bandwidth ultrasensitive molecular detections.
To accomplish these advances, our group spent several years studying comb generation and developing precision control of nonlinear spectroscopy based on frequency combs. We developed the capability to synchronize the pulse trains from two independent femtosecond lasers and phase lock their carrier frequencies, establishing phase coherence between the two lasers, as reported in Science in 2001. We also used the same two lasers generate pulses in the mid and far infrared and experimented with precisely controlling both pulse timing and the carrier-envelope phase. Together with research into passive optical cavities, this research led to improvements in linear and nonlinear spectroscopy and coherent control. In 2005, our work on frequency combs led to the creation one of the world's first two VUV frequency combs; the other was generated at the Max-Planck-Institute of Quantum Optics in Garching, Germany. Today, our group continues to work on improving the range and capabilities of frequency combs as part of its ongoing research on laser stabilization and comb characterization.
In less than a decade, optical frequency combs have revolutionized optical frequency metrology and synthesis. They are the basis of optical atomic clocks under development in the Ye labs and around the world. Today, frequency combs provide a precise means of laser pulse manipulation and control, enabling coherent pulse synthesis and distribution. As a result, researchers have begun to explore completely arbitrary optical-waveform synthesis, an ability that both complements and rivals similar technologies developed in the radio frequency domain. Frequency combs are also helping researchers push the frontiers of phase-sensitive nonlinear optics. By pursuing a united approach in time- and frequency-domain controls (see direct frequency comb spectroscopy), our group is now pursuing simultaneous coherent control of quantum dynamics in the time domain and atomic and molecular structure in the frequency domain.
Direct Frequency Comb Spectroscopy
Optical frequency combs have proven to be an excellent and low-cost tool for precise studies of atomic and molecular structures. In direct frequency comb spectroscopy, for example, researchers use light from a comb to measure electron energy levels in atoms, detect and control atomic energy level transitions in real time, investigate quantum coherence, study atomic and molecular dynamics, and even identify unknown atoms and molecules by detecting and identifying the characteristic energy level transitions. Our group has used comb-based atomic spectroscopy of ultracold rubidium atoms to precisely determine atomic transition frequencies and control transition pathways as well as to develop molecular spectroscopy for ultrasensitive detection of trace molecules.
Atomic spectroscopy of ultracold rubidium
Until 2004, ultrashort laser pulses were used in two distinct modes, the frequency domain or the time domain. In the time domain, researchers used them to investigate and manipulate atoms on subpicosecond time scale. In the frequency domain, researchers used optical frequency combs produced by stabilized mode-locked lasers as an absolute frequency reference across wavelengths ranging from the microwave through the visible. A singular achievement of the Ye labs was to combine these two applications in spectroscopic studies of one- and two-photon transitions in laser-cooled 87Rb atoms. To accomplish this, the group used a phase-stabilized femtosecond pulse train from a mode-locked laser that produced a spectral resolving power comparable to that of the best continuous-wave lasers. In so doing, they bridged the fields of high-resolution spectroscopy and ultrafast dynamics, creating a "united time-frequency spectroscopy" for the study of the structure and dynamics of atoms (and now molecules).
The new method used the laser pulse train to precisely measure the electron energy levels of 87Rb atoms and detect and control several different transitions between the natural, or ground-state, energy level to two or three other higher energy levels as fast as they occurred. Different frequency comb components were encoded and used to control both constructive and destructive quantum interference effects in the transitions. The ultrashort pulses also made it possible to precisely determine the fraction of atoms in each energy state and track how those populations changed with time. To track the changes, we measured the fluorescent light produced when excited atoms relaxed back into their ground state. The scientists also realized that transition amplitudes by successive pulses could add up coherently over time, making it straightforward to fine-tune specific energy-level transitions using less laser power than expected. In essence, the energy contained in each individual pulse could be small to avoid undesired effects in atoms or molecules, but the collective effect of multiple pulses working together coherently would achieve targeted responses at high efficiency.
At the same time, we were able to use the narrow comb teeth to precisely and efficiently determine all the natural electron energy levels in 87Rb atoms in the optical, terahertz, and radio-frequency spectral domains. We also investigated the interaction of the comb with the atomic gas and learned how to precisely control these interactions. We were able to sufficiently reduce the technique's systematic errors to create a new precision spectroscopy, known as direct frequency comb spectroscopy.
Since the initial direct frequency comb spectroscopy experiments, our group has continued to investigate the structure and dynamics of cold 87Rb atoms. Using pulse-shaping techniques to change the phase of the different colors of the pulse train, we have investigated different two-step pathways electrons can travel between the ground state and a specified excited state. By using selection rules, we ensure that the different electronic transitions go through a single intermediate state to the same final state. Our group has observed that not only do the amplitudes of photon pairs interfere with each other, but interference can also occur with single electron wave functions. We've shown that the highest resolution in determining a particular transition frequency occurs when many pulses are used. Many pulses interfere with each other, washing out everything except a narrow frequency band at the ideal electronic transition.
Recently, our group began investigating multilevel four-photon transitions in cold 87Rb atoms. By linking one comb tooth (frequency) to each electron pathway, we've been able to control the phases of the different pathways. We've even been able to detect and control interference between different transition pathways, a phenomenon they continue to explore.
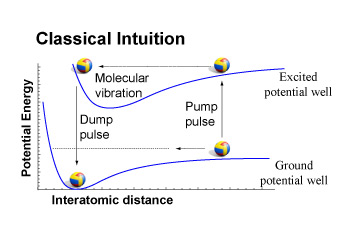
A classical representation of the pump/dump method for
transferring a highly excited Feshbach molecule into a
stable ground state.
In related efforts, we've completed a theoretical analysis of a new frequency comb-based method for producing stable, deeply bound cold polar molecules from large, loosely bound, and relatively unstable cold molecules formed from cold atoms via magnetic Feshbach resonances. The new method can be intuitively understood classically.
Although strongly vibrating Feshbach molecules oscillate back and forth through a potential well, their intense vibrations cause them to mostly stay near the external turning point of the potential well. Because of this behavior, it's possible to use ultrashort laser pulses to pump Feshbach molecules into new, excited electronic potential wells, where their nuclei begin to vibrate in response to their new environment. If the inner turning point of the new potential well sits directly above the lowest point in the ground potential well, then another laser pulse can dump the molecule directly to the bottom of the ground well, i.e., into its ground state. It's all a matter of timing: The dump pulse must occur when the molecule is vibrating at the inner turning point of the excited well.
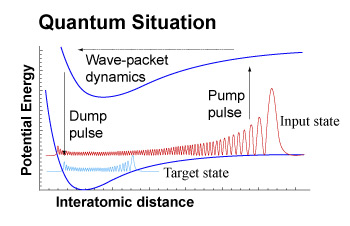
On the quantum mechanical level, the pump/dump process occurs
in many small steps, which each transfer a small fraction of
the molecular wave function.
Quantum mechanically, this process occurs in many small pump/dump steps. With each step a small fraction of the molecule's wave function is transferred to the ground state. The cumulative effect of many laser pulse pairs, or coherent accumulation, is what drives the molecules into the ground state. However, the process can only work if the pump and dump pulses are perfectly synchronized to the behavior of the molecules. This synchronization is accomplished by having both the pump and dump lasers be femtosecond frequency combs with exactly matching teeth (frequencies). Even with the combs, coherent accumulation requires time during which the molecules must not be disturbed by collisions. Hence, the method is well suited for cold matter where coherence times are long.
We're currently preparing a series of experiments (in collaboration with the Jin group) to test the pump-dump theory against continuous-wave laser techniques for producing stable, ground-state Feshbach molecules of KRb. We anticipate that our research on frequency comb-based control strategies will lead to the production of ultracold molecules from pairs of ultracold atoms.
Molecular spectroscopy: ultrasensitive, high-resolution detection across a broad bandwidth
In 2006, two years after the invention of direct-frequency comb spectroscopy, our group developed the powerful new variation of cavity-enhanced direct frequency comb spectroscopy. The new method allows the group to study molecular vibrations, rotations, and collisions as well as temperature changes and chemical reactions. It also can detect trace amounts of unknown chemicals with exquisite sensitivity because it can identify characteristic patterns of laser-light absorption, or molecular fingerprints. The broad spectral bandwidth of the comb makes it possible to detect millions of parallel light channels simultaneously in real time.
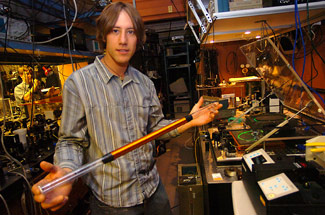
Mike Thorpe with the optical cavity he created to implement
cavity-enhanced direct frequency comb spectroscopy
In this method, an ultrafast laser creates an optical frequency comb spanning visible and near infrared wavelengths and couples it into an optical cavity containing unknown atoms and molecules. Coupling ensures that the light in the cavity maintains the same precise frequency comb and dramatically enhances the interaction length between the light and the intracavity medium. The chemicals inside the cavity absorb photons of particular frequencies. These absorption patterns are unique to each atom or molecule. Thus when light exits the cavity, it can be analyzed to see not only what frequencies of light are missing photons, but also how many photons of a particular frequency have disappeared. Since the pattern of missing photons is unique for each chemical, the unknown atoms and molecules can readily be identified via comparisons with known molecular fingerprints of individual constituents.
Since the initial demonstration of this technique, our group has developed a fiber laser for molecule detection that has orders of magnitude better sensitivity because its light is a better match with characteristic molecular absorption spectral regions. In a recent trace-gas detection experiment, the group used a near infrared comb spanning wavelengths from 1.5 to 1.7 µm to rapidly and precisely identify 10 different molecules in a gas. The group has also used its refined method to differentiate among isotopes of molecules of CO and C2H2. The refined method could potentially identify trace amounts of hundreds or even thousands of different chemicals.
The group expects applications of cavity-enhanced direct frequency comb spectroscopy to appear soon. The applications include (1) medical breath analysis for diagnosing illness by detecting telltale chemicals signaling infections, diabetes, kidney disease, cystic fibrosis, or other diseases, as well as (2) security screening for potential hazards such as explosives, toxic gases, or dangerous pathogens. These applications will benefit from cavity-enhanced direct-frequency comb spectroscopy's ability to detect trace amounts of chemicals (less than one molecule out of 1 billion).
Earlier we developed an even more sensitive "optical nose" technique that uses an infrared laser and an optical cavity with highly reflective mirrors to identify trace amounts of chemicals. The optical nose can identify one molecule among 1 trillion others. However, this technique works less well than direct-frequency comb spectroscopy for identifying components of unknown mixtures because it can analyze only one frequency of light at a time.
With ultrasensitive detection of trace chemicals coming along nicely, our group recently turned its attention to advanced molecular beam spectroscopy. Molecular beam spectroscopy uses the trace detection principles developed for cavity-enhanced direct-frequency comb spectroscopy to gather broadband information on the quantum states of a beam of cold molecules. It enables high-resolution measurements of rotational and transverse molecular temperatures and of state-dependent concentrations as functions of position. Simply put, it's a precise and powerful application of an infrared frequency comb (1.5-1.7 µm and beyond) to the study of cold molecules. We expect it to become a wonderful tool for characterizing cold molecules, detecting their presence, and studying the dynamics of ultracold chemical reactions.
XUV Comb Generation
In 2002, our group decided to apply an old idea for amplifying and stabilizing continuous-wave (cw) lasers to ultrafast mode-locked lasers. In less than three years, these efforts yielded one of the first two frequency combs in the vacuum ultraviolet (VUV). Our group's insight was to modify the build-up cavities used to amplify cw laser outputs to work with the ultrafast lasers. Within a year, we had succeeded in coherently enhancing pulse energies inside an optical cavity by a thousandfold, while maintaining the high quality of the original laser pulses. This achievement opened the door to generating coherent, high-repetition frequency light in VUV wavelengths. The power enhancement provided by the femtosecond enhancement cavity has also allowed us to study extreme nonlinear optics without expensive femtosecond amplifiers.
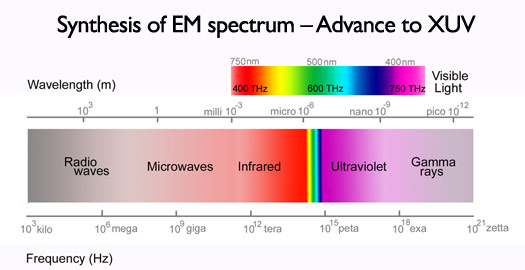
refinement of a high-finesse cavity and its custom mirrors.
The secret ingredient to creating the new high-finesse cavity was custom-designed mirrors. The high-broadband mirrors were highly reflective and exhibited low-controlled dispersion. They made it possible to produce highenough peak intensities inside the cavity to ionize such noble gases as xenon, krypton, and argon. Eventually, the mirrors were able to keep light pulses circulating through these gases for hundreds of round trips, generating shorter and shorter wavelengths of coherent light.
Our group then used this precision high-finesse optical cavity with an ultrafast mode-locked laser (emitting in the near infrared) to generate a VUV frequency comb. The infrared laser pulses were enhanced nearly a thousandfold inside the cavity, which allowed high harmonic generation into the VUV at 100 MHz repetition rates. The VUV comb is a short-wavelength version of the optical frequency combs that have revolutionized optical frequency metrology, precision measurement, and optical pulse synthesis and control, including demonstrations of optical atomic clocks. The creation of the VUV comb has opened the door to conducting coherent light-based experiments in VUV. This capability will allow researchers to investigate the fine structure of atoms and molecules and make ultraprecise measurements of differences in the energy levels of light emissions, the timing of chemical reactions, or dimensions of nanometer-sized objects.
Since it created its first VUV comb, our group has been investigating ways to increase the power of the system without damaging the enhancement cavity mirrors. First, we figured out how to maintain the plasma inside cavity for several hours before the mirrors burn — a substantial increase in time from the tens of seconds the mirrors lasted at first. Second, the group has substituted a fiber mode-locked laser for a Ti:sapphire mode-locked laser in the comb generator. We have already successfully used the fiber laser to scale the power level of the enhancement cavity by tenfold. Third, the group has determined that argon is better than other noble gases for high harmonic generation in the enhancement cavity. Finally, we are working on better methods for getting VUV, and eventually XUV, combs out of the cavity once they are created.
The group has already made important progress in scaling the peak intensity of the VUV comb generator and significant improvements in the reliability and reproducibility of the system, opening the door to precision metrology in the VUV region. Once the VUV comb generator is optimized, our group plans to perform precision spectroscopy on cold neutral helium atoms, an extremely challenging task that requires VUV light.
Laser Stabilization & Comb Characterization
JILA's role in the discovery and implementation of optical frequency combs grew out of a long history of leadership on continuous-wave (cw) laser stabilization research by Nobel Laureate Jan Hall, who has now retired. The application of Hall's cw laser stabilization techniques to ultrafast mode-locked lasers paved the way to making optical frequency combs ultrastable and the journey of discovery our group embarked upon eight years ago. Since then, we have explored and expanded optical frequency comb technology in ways we only dreamed of back then. Today we use frequency combs to investigate the structure of and control the dynamics of cold atoms and molecules, build ever-more precise and accurate optical atomic clocks, identify unknown chemicals, and make extraordinarily precise measurements of frequency and length.
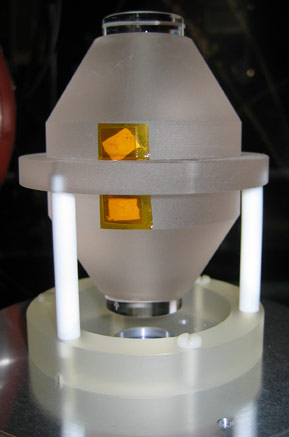
This vertically mounted high finesse optical cavity
signifantly increases the stability of a laser system
using a fiber laser.
To develop the best possible tools for these investigations, our group maintains a robust research program in laser stabilization and comb characterization. The goal of current laser stabilization work is to make the optical phase (cycle of peaks and troughs) coherence last more than 10 s. To accomplish this, we usually servo lock the laser output to an isolated passive optical cavity and use multiple techniques to reduce cavity noise due to mechanical changes, vibrations or temperature fluctuations.
For example, our group recently developed a new, compact high-finesse optical cavity that significantly reduces vibration sensitivity. A cavity-laser system consisting of the new cavity and a diode laser is extremely stable. Several innovations are responsible for this stability, including (1) a vertical mounting configuration, (2) a relatively short cavity length, (3) a shape with a wide center and narrow ends, and (4) a midplane attachment to its base. The cavity-stabilized laser has a subhertz linewidth (at several seconds) and a fractional instability of ~1 x 10-15 at time scales of 0.5-300 s. Our group recently used the laser to resolve an optical transition in atomic strontium of ~2 Hz, the highest quality factor ever observed.
Our group anticipates using the new laser system for precision and quantum measurements. In the future, we plan to push new lasers to even higher stability levels. To accomplish this, we will investigate ways to further limit thermal noise in optical cavities, including cooling the cavities, varying the cavity length, and investigating improved cavity-spacer and coating materials.
In related research, our group continues efforts begun in 2000 to characterize octave-spanning optical frequency combs. We count many successes since this effort began. For example, we have learned to directly measure and precisely control the two parameters that characterize an optical frequency comb in the frequency domain: the pulse repetition frequency (ƒr) and the carrier-envelope offset frequency (ƒ0), which arises from pulse-to-pulse variation in the carrier-envelope phase. We have demonstrated stable transfer of optical frequency standards across huge frequency gaps to other optical spectral regions and shown how this optical stability can be transferred down to the microwave region. Transfers of precise optical frequency combs over many kilometers of fiber network have also demonstrated the potential of delivering multiple stable frequency references at once. We have also created a stable network of microwave and optical frequencies that surpass previously known technologies and cost far less to implement. Our group now routinely uses an optical frequency grid with hundreds to hundreds of thousands of comb lines repeating every 100 MHz - 1 GHz over a bandwidth of an optical octave, with every line stable below the 1 Hz level.
At the same time, our group has worked to enhance experiments and precision measurement in the time domain. We've stabilized the carrier-envelope phase of mode-locked laser pulse trains at a level of tens of milliradians. We've synchronized two femtosecond pulse trains and phase-locked their carrier frequencies, establishing phase coherence between two lasers. Using the same two lasers, we've been able to generate pulse trains the mid and far infrared, in the process extending frequency combs into this region and opening the door to coherent control of molecular systems.
Today, we apply optical frequency combs to precision spectroscopy, absolute frequency metrology, optical atomic clocks, and optical frequency synthesis. We also use pulse-timing synchronization and distribution1, coherent pulse manipulation, and direct frequency comb spectroscopy. All this is possible because we can now control the femtosecond comb as precisely as any continuous-wave laser. However, our group seeks to do even better.
We are currently testing the fundamental properties of an octave-spanning frequency comb to see how faithfully the comb transfers its coherence properties. We want to determine the technical limits of the comb and the fundamental theoretical explanations for these limits. We plan to lock the comb to one part of the spectrum and precisely measure the linewidth of comb lines an octave apart. We plan to compare the higher frequency line with the value calculated from a measurement of the lower frequency line. Because optical frequency combs have entered the realm of precision measurement at the highest level, our group wants to determine the characteristics of the comb at the most precise level that is possible experimentally.
Lab temperature data logger
Lab status: 128.138.107.209
Lab status: 128.138.107.217